Functional ultrasound (fUS) imaging
Pioneering 4D ultrafast cardiac imaging
Our team has built the very first prototype for 4D ultrafast ultrasound imaging, capable of imaging the human heart at several thousands of frames per second, and to quantify a complete set of functional parameters: the myocardium contractility, the electrical activation and the blood flow can be recorded during a single heartbeat. These data will ultimately strengthen the diagnosis of many cardiovascular diseases.Our work here includes observing heart valve-associated electromechanical vibrations and demonstrating the feasibility of semi-automatic evaluation of cardiac Doppler indices in a single cardiac cycle (Papadacci et al., 2020 IEEE TMI; Papadacci et al., 2019, Physics in Medicine & Biology).
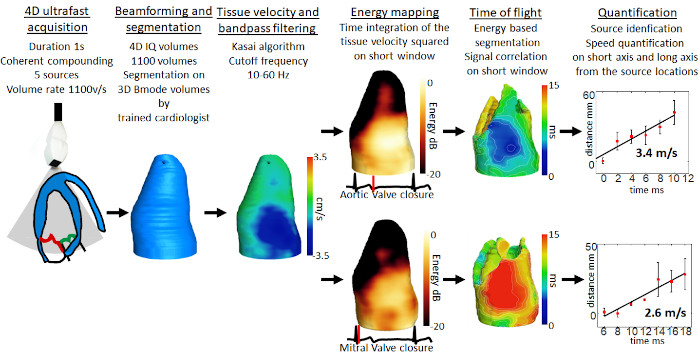
Functional ultrasound brain imaging: a new paradigm for neurosciences
Ultrasound is entering the world of neurosciences with the use of ultrasensitive Doppler techniques to detect small blood flow variations induced by neuronal activation. With neurofunctional ultrasound imaging, the brain activity can be imaged with an excellent spatio-temporal resolution and an exquisite sensitivity, embedded in portable bedside systems.
At the institute Physics for Medicine Paris, we are shedding light on the new role of functional ultrasound imaging in neuroscience. This revolutionary technique introduced by our lab in 2010 allows to study the function and connectivity of different organs within the central nervous system, including the brain, retina, and spinal cord.
We have successfully applied it to research on various behavioring active subjects, including rats, mice, ferrets, and non-human primates with a successful industrial transfer to the startup Iconeus and we are exploring clinical applications in humans.
- Awake functional brain imaging
- Whole-brain functional connectivity in awake mice
- Spinal cord, ganglions and retina functional imaging
- Functional ultrasound imaging of the brain in clinics
- Brain-Machine Interfaces
- Characterizing alterations in neurovascular coupling
- Functional ultrasound localization microscopy (fULM)
- Stroke imaging and monitoring
- Hybrid PET-Ultrasound imaging
Awake functional brain imaging
We are exploring imaging in awake and behaving subjects.
In non anesthetized rats, we studied locomotion and sleep with a small implantable fUS probe. We observed significant hemodynamic adaptation in the brain, an occurrence that relates to the adjustment of blood flow within the brain(Bergel et al., 2020, Nature Communications). We further identified strong hemodynamic activity during REM sleep, prompting questions about the evolutionary advantage of such an energy-consuming event during sleep (Bergel et al., 2018, Nature Communications). Moreover, we discovered that the posterior amygdala, an area of the brain related to emotional processing, operates independently from other brain regions during REM sleep, which suggests its potential involvement in emotional regulation and dream content during this sleep stage.
We explored rapid 3D tonotopic maps in awake ferrets with Yves Boubenec’s team at ENS. This development allows us to visualize the functional organization of sensory systems in unprecedented detail (Bimbard, 2019, ELife). We also partnered with Pierre Pouget’s team at the Brain and Spine Institute and Serge Picaud’s team at the Vision Institute, using our fUS technology to image the activity in various regions of the brain in awake primates during cognitive and visual tasks (Dizeux et al., 2019, Nature Communications; Blaize et al., 2020, PNAS).
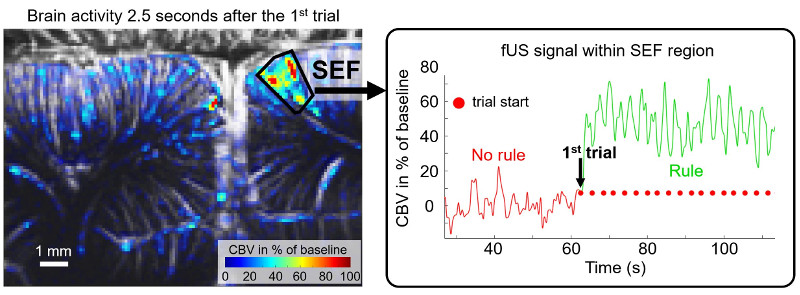
Whole-brain functional connectivity in awake mice
Mice are a key model for biomedical research due to the availability of diverse genetic variants. In our institute, we have achieved significant strides in whole-brain functional transcranial ultrasound imaging in awake mice. We have implemented strategies to automate positioning of regions of interest through a brain navigation tool and to detect drug-induced changes in brain functional connectivity, establishing valuable biomarkers for drug evaluation (Tiran et al., 2017, Ultrasound in Medicine & Biology; Nouhoum et al., 2021, Scientific Reports; Rabut et al., NeuroImage, 2020).

Furthermore, together with Zsolt Lenkei’s team at the Institut de Psychiatrie et Neurosciences de Paris, we have explored the existence of the default mode network in awake mice, a network previously observed only in humans, primates, and rats (Ferrier et al., 2020, PNAS). Functional connectivity studies have also provided insights into neurodevelopmental changes in offspring of mothers with protein-deficient diets (Mairesse et al., 2019, Glia) and changes in connectivity associated with chronic pain (Rahal et al., 2020, Scientific Reports). We now work on drug fingerprints using functional connectivity.
What are Drug fingerprints ?
Functional brain connectivity matrices are a way to measure how correlated two regions’ activity are. Blood flow to different regions can be traced, and when two regions are active at the same time, it is assumed they are interacting. These interactions can be presented as matrices :
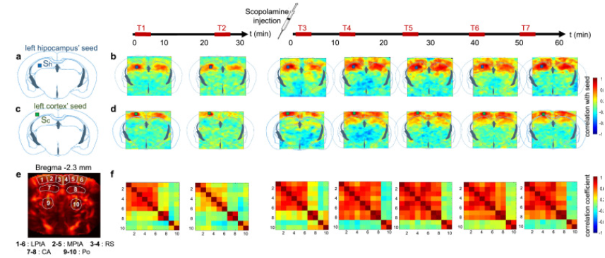
A drug’s “fingerprints” are a score of the pharmacological effects of a drug on the brain.
fUS allows the quantification of blood flow variation in the brain with high sensitivity, which in turn tells us about brain and neuronal activity, and how it changed after the drug was given to the patient. They are called fingerprints as they will leave distinctive marks on the functional connectivity matrices.
fUS can therefore be used to study a drug’s effect on the brain, with its edge over MRIs being that it doesn’t require the test subject to be asleep and consequently, can yield results free from anesthesia-induced bias. It is also able to map out the entire brain in one imaging session, which helps researchers assess the effects of a drug on the entire brain in real time.
See also : https://iconeus.com/pharmaco-fus-is-here/
Spinal cord, ganglions and retina functional imaging
Beyond the brain, we’ve expanded our exploration of functional imaging to different organs. We’ve utilized our technology to study the specificity of hemodynamic responses in the rat spinal cord to different types of stimulation (Claron et al., 2021, Pain). Additionally, we’ve explored the vascularization of the trigeminal ganglion and its activation during facial stimulation (Réaux-le-Goazigo et al., 2022, Communications Biology).
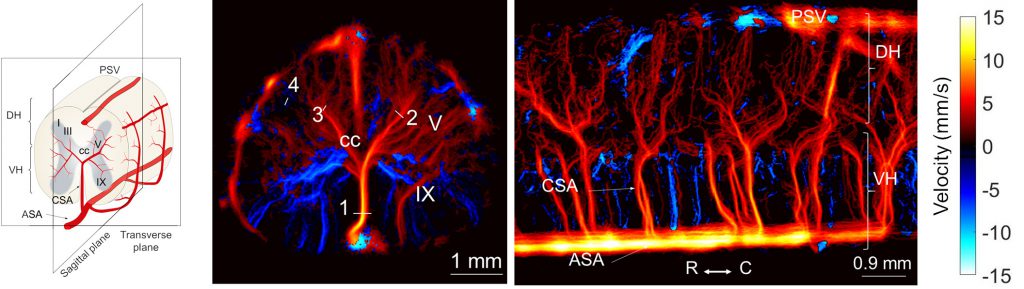
In the rat retina, we have proven fUS to be a promising tool for early detection of neurovascular alterations in Alzheimer’s disease models (Morisset et al., 2022, Scientific Reports).
Functional ultrasound imaging of the brain in clinics
In our clinical work, we have successfully deployed intraoperative functional ultrasound imaging (fUS) for cortical mapping in patients with low-grade gliomas, allowing us to identify functional areas related to various motor and sensory tasks (Imbault et al., 2017, Scientific Reports).
Additionally, we have performed several clinical studies in collaboration with the hospital Robert Debré, to demonstrate fUS in newborns as a tool to detect sleep states, identify epileptic foci, and analyze brain functional connectivity (Demené et al., 2017, Science Translational Medicine; Baranger et al., 2021, Nature Communications). These studies underscore the potential of fUS for early identification of abnormal brain development.
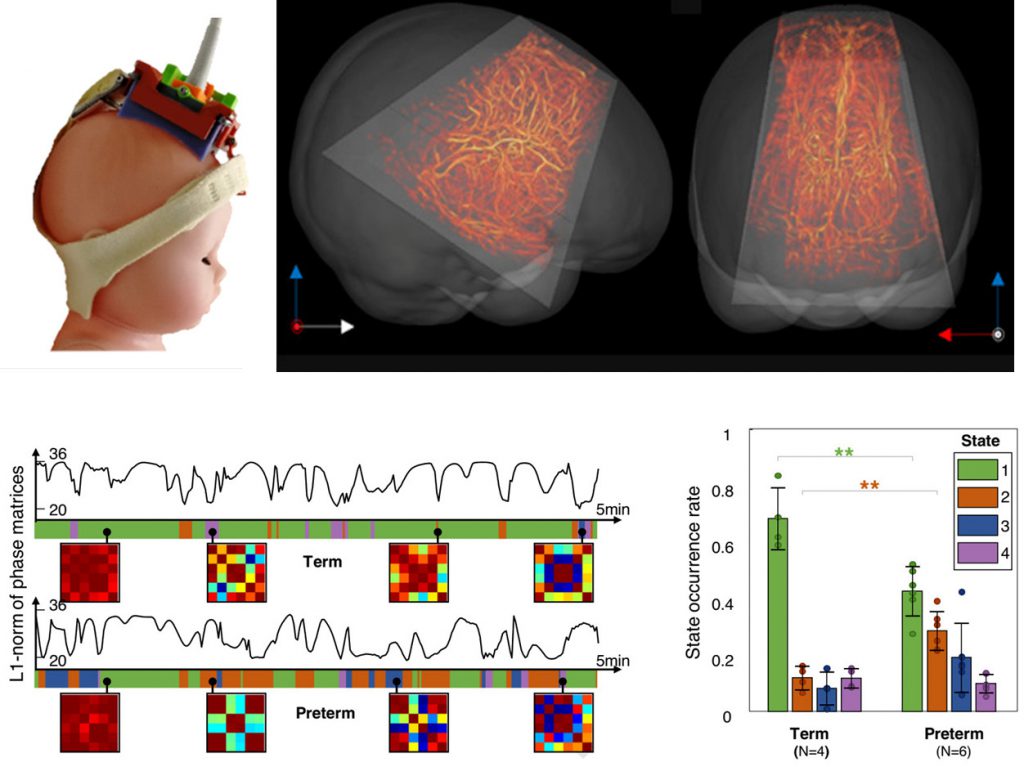
Brain-Machine interfaces
In collaboration with the California Institute of Technology, we demonstrated the feasibility of a functional ultrasound-based brain-machine interface (BMI), a novel application of fUS technology that could mitigate the limitations of traditional BMIs (Norman et al., 2021, Neuron)
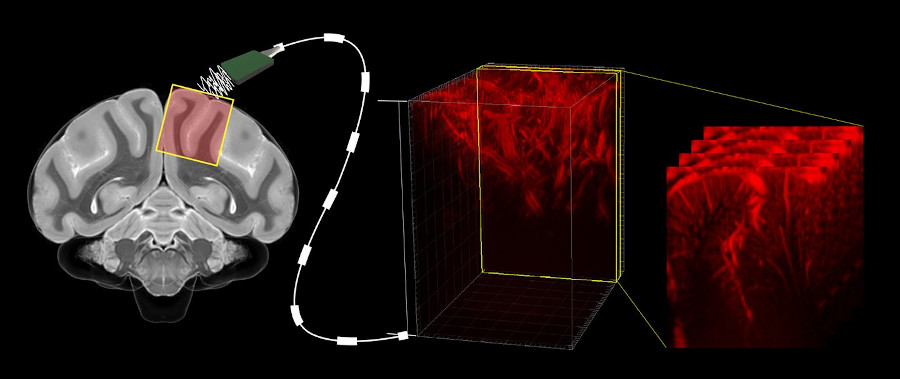
To understand neural activity, and to diagnose and treat neurological diseases (like Epilepsy, Parkinson’s, Alzheimer’s…), we often use electrophysiology, which requires the implantation of electrodes via open brain surgery, a dangerous and complicated operation. Other non-invasive methods, such as electroencephalography and functional MRIs, have also been successful, although they are limited by the dispersion of signal through various tissues including bone as well as low spatial resolution of summing activity from large brain volumes.
fUS can be used in a minimally invasive way, providing excellent spatiotemporal resolution and high sensitivity over large fields of view. Unlike the MRI, it can be used on moving subjects, which allows us to study brain activity in awake animals. For example, we were able to detect movement intentions in primates.
As a result, such technology could have a large impact on the development of neuroprosthetics, which can drastically improve the lives of patients with impaired nervous systems, allowing them more agency and autonomy in day-to-day life.
Characterizing alterations in neurovascular coupling
Neurovascular coupling describes the close interaction occuring in the brain between neurons and blood vessels, and supporting the brain functioning. We have developed a protocol to characterize neurovascular coupling alterations in mouse models with small vessel diseases, an approach that could help to diagnose cerebrovascular dementias at an early stage. We are also exploring the possibility of translating this approach to human patients by assessing these alterations in the retina (Morisset et al., 2022, Scientific Reports).
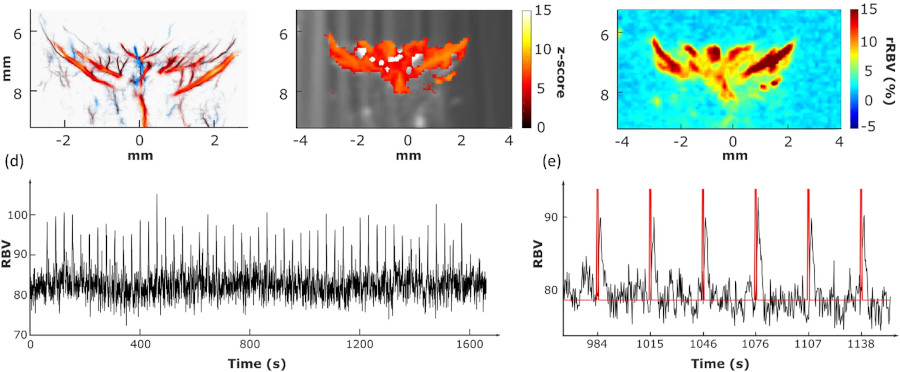
In diseases like Alzheimer’s, the symptoms can be detected through MRI’s and CT scans. However, by the time we are able to detect them with imaging, the tissues often have already been damaged, and treatment is unlikely to have a significant impact on the patient’s condition.
In neurodegenerative diseases, neurovascular coupling impairments have been shown to be early signs of disease, occurring up to years prior to the first symptoms. On top of that, the retina is formed from the same tissue as the brain is during embryological development, and has similar needs in terms of glucose and oxygen to the cortex. Thus, analyzing neurovascular coupling in the retina shows much potential in early diagnosis and better treatment of many neurodegenerative diseases such as Alzheimer’s.
MRIs being ill-suited for early diagnosis measures such as these, it is believed fUS, a cheaper and lighter alternative, could be the way forward in order to detect neurovascular coupling alterations in patients as a preventative screening method for dementia.
Functional ultrasound localization microscopy (fULM)
We’ve recently developed a novel method of functional neuroimaging called functional ultrasound localization microscopy (fULM), which merges fUS technology with super-resolution microscopy to map local brain activity at an unprecedented level of detail (Renaudin et al., 2022, Nature Methods). This pioneering work opens up a new field in neuroscience research, allowing us to study vascular dynamics throughout the entire brain at a microscopic scale.
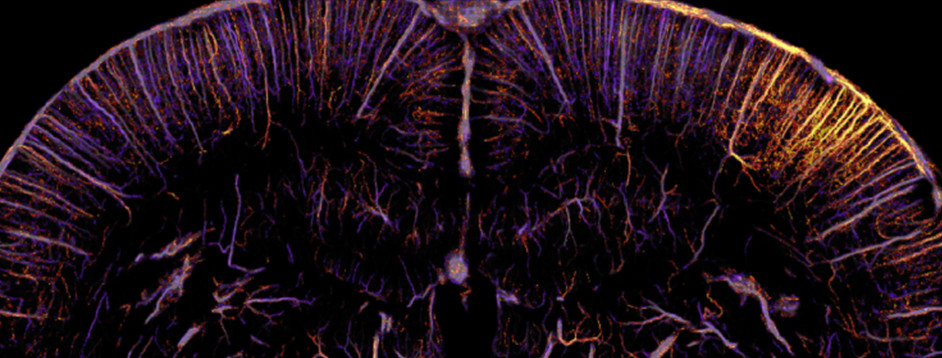
Stroke imaging and monitoring
Our work on ischemic stroke in mouse models with Denis Vivien’s team at the GIP Cyceron on post-cardiac arrest models in rabbits has demonstrated the potential of fUS to study neuroprotective mechanisms and therapeutic strategies (Hingot et al., 2020, Theranostics; Demené et al., 2018, Scientific Reports).
Currently, the standard method used for cerebral imaging is the MRI. While it allows for precise diagnosis of brain injuries, it is expensive and hard to transport. On the other hand, the fUS devices are a lot smaller and thus easier to move and store, as well as being cheaper. They also manage to combine several interesting attributes of other methods, such as repeatability, being able to be used at bedside, and providing both quantitative measurements and measurements on multiple perfusion parameters.
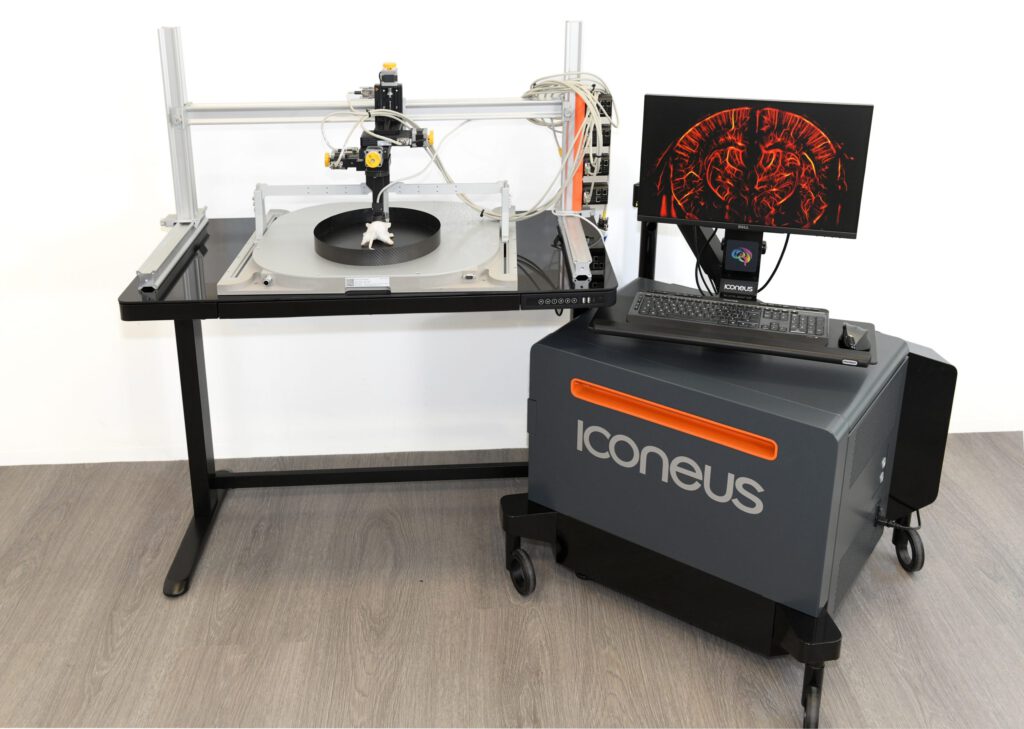
Typical set up for mouse brain imaging with a fUS device.
After a stroke, recanalization and reperfusion of the brain are the major factor in prognostics of a good outcome for the patient. Thanks to the fUS, researchers were able to monitor the restoration of the perfusion within the first 2 hours post-stroke, and identify which regions were hypoperfused in mice’s brains.
At 24 hours post stroke, it was revealed via MRI that these same identified regions were those suffering lesions.
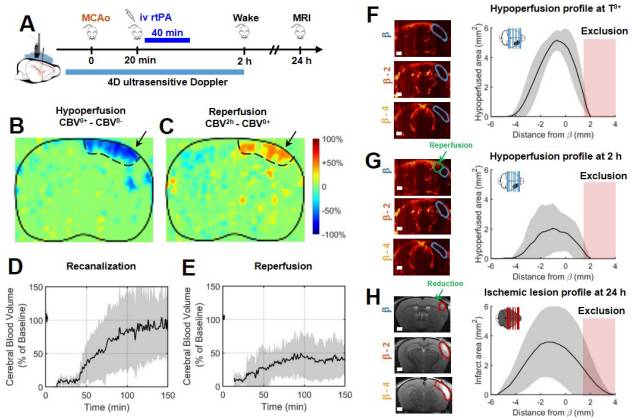
The data the fUS device provides is very beneficial in identifying how the lesions extend over time after a stroke, which is an important goal in stroke therapy. Research is still on-going in order to apply this technique to humans.
Hybrid PET-Ultrasound imaging
We have developed the world’s first preclinical multimodal PET-CT-Ultrasound system in collaboration with Bertrand Tavitian’s team at HEGP. This system enables simultaneous imaging of metabolic and vascular activity in rodents during tumor growth (Provost et al., 2018, Nature Biomedical Engineering; Perez-Liva et al., 2020, Molecular Imaging and Biology).
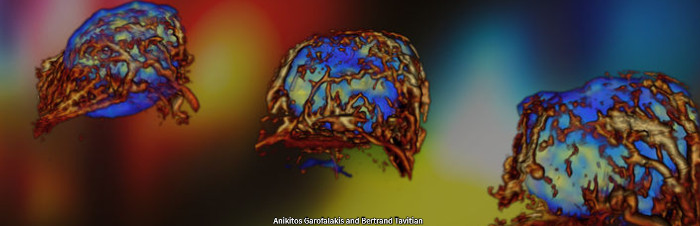
Positron emission tomography – computed tomography (or PET CT) is the most sensible molecular imaging modality. However, motion significantly decreases the quality of the images it can produce, which has been a problem when it came to imaging the heart, especially in a research context on rodent hearts. On the contrary, ultrafast ultrasound imageging is fully capable of capturing a large amount of high quality images of live mice hearts in real time.
Combining both technologies has allowed for an easier identification of anatomical lesions, as well as their metabolic consequences in the heart.